1. INTRODUCTION
Bulk heterojunction (BHJ) organic solar cells have heavily relied on fullerenes such as phenyl-C61-butyric acid methyl ester (PC61BM) and phenyl-C71-butyric acid methyl ester (PC71BM) for the past two decades, with their unrivaled electron-acceptor properties: good solubility in organic solvents, high electron mobilities, low-lying energy levels, and their ability to form nanoscale internetworked domains for efficient charge separation [1-4]. However, fullerene electron-acceptor molecules suffer from several drawbacks, such as weak absorption in the visible light region of the solar spectrum, poor tunability in terms of energy level, and morphological instability over time. In recent years, great efforts have been devoted to developing non-fullerene electron acceptors (NFAs), driven by the need to overcome the intrinsic limitations of fullerene acceptors [5-8].
Rapid progress has been made for NFA-based organic photovoltaic (OPV) devices over the past few years: numerous studies reported outstanding power conversion efficiencies (PCEs) of over 17% for single junction OPVs, which far exceeds the PCEs of fullerene-used devices [7,9-12]. This fact demonstrates that NFAs have great potential for future applications in organic solar cells. The reasons for the advanced performance of the NFA-based solar cells are as follows. The energy levels of NFAs can be easily tuned by systematical modification of molecular structures, which allows for achieving a high open circuit voltage (Voc) when combined with various choices of well-developed electron-donor materials [13-17]. NFAs have strong near-infrared (IR) light harvesting capability and comprise a complementary broad range light absorption when they are blended with high bandgap polymer donors, which would be beneficial to increasing the short circuit current (Jsc). In addition, planar NFA molecular backbones increase the intermolecular interactions with an appropriate crystalline domain size in the photoactive layer, enabling efficient charge carrier transport [7],[17-20]. There are also some reports that the morphological stability for NFA-based OPVs is better than that of the fullerene acceptor-based OPVs [21-25].
The relationship between the molecular structure of NFAs and material properties should be understood to properly design an effective electron-acceptor molecule for high-performance organic solar cells. NFAs are typically designed to have a planar structure composed of alternating electron-donor (D) units and electron-acceptor (A) units [13-14,21-24,26-29]. Symmetrical A-D-A or D-A-D backbone structures with well-designed alkyl side chains enable proper self-assembly through intermolecular interactions between the backbone structures or between the alkyl side chains, thereby improving crystallinity and increasing long-range molecular order in thin films. Moreover, the linkage of A or D chemical moieties dictates light absorption and energy level alignment of the highest occupied molecular orbital (HOMO) and the lowest unoccupied molecular orbital (LUMO) levels of the NFAs to polymer donors for high photovoltaic properties. It is also well known that the fluorination of molecular backbones improves OPV device performance due to the following reasons: (i) fluorination simultaneously down-shifts the HOMO and LUMO levels. Thus the molecular bandgap energy remains the same [30-31]; (ii) the small size of fluorine barely causes steric hindrance; (iii) non-covalent interactions such as F⋯H and F⋯S interactions enhance intramolecular or intermolecular interactions, increasing crystallinity [31-35]; and (iv) fluorinated semiconducting molecules often show higher absorption coefficients compared to their nonfluorinated counterparts [26-27,36].
In this work, we investigated the effect of systematical modifications in the backbone structures or alkyl side chains on NFA material characteristics and photovoltaic properties. As seen in Fig. 1, all molecules were designed by employing intramolecular F⋯H, N⋯H, F⋯S, or N⋯S interactions to ensure the planarity of each molecule [31-35]. The DFD series (the chemical structures of DFDO-RC2 and DFDE-RC2 are shown in Fig. 1(a) and Fig. 1(b)) molecules consist of electron-deficient difluorobenzothiadiazole (F) as a core, electron-rich dithienosilole (D) as a π-conjugated bridge, and benzodiathiazole-connected 3-ethylrhodanine (RC2) units at each end. By employing two types of alkyl chains, octyl (O) and 2-ethylhexyl (E), as alkyl side chains, we intended to study how alkyl side chains affect intermolecular organizations. In the PTB7-Th:DFD-RC2 based OPV devices, the HOMO-HOMO energy offset (≤0.09 eV) between the DFD-RC2 materials and PTB7-Th was insufficient, which resulted in low PCE values (≤1.30%). Thus we replaced difluorobenzothiadiazole (F) with difluorobenzene (FBz) moiety to achieve enough HOMO-HOMO offset. The resulting DFBz series (the chemical structures of DFBzO-RC2 and DFBzE-RC2 are shown in Fig. 1(c) and Fig. 1(d)) molecules have indeed lowered HOMO levels and thus increased the HOMO-HOMO energy offsets between DFBz-RC2 molecules and PTB7-Th. Moreover, the extinction coefficients and crystallinity of the DFBz series were improved. DFBzE-RC2 exhibited optimal phase separation with PTB7-Th and showed interconnected nanoscale domains, whereas DFBzO-RC2 showed excessive phase separation with PTB7-Th. As a result, the highest Jsc and fill factor (FF) values were observed in PTB7-Th:DFBzE-RC2 device. We also note that a remarkably low energy loss of 0.51 eV and high Voc of 1.07 eV was attained in the PTB7-Th:DFBzE-RC2 device.
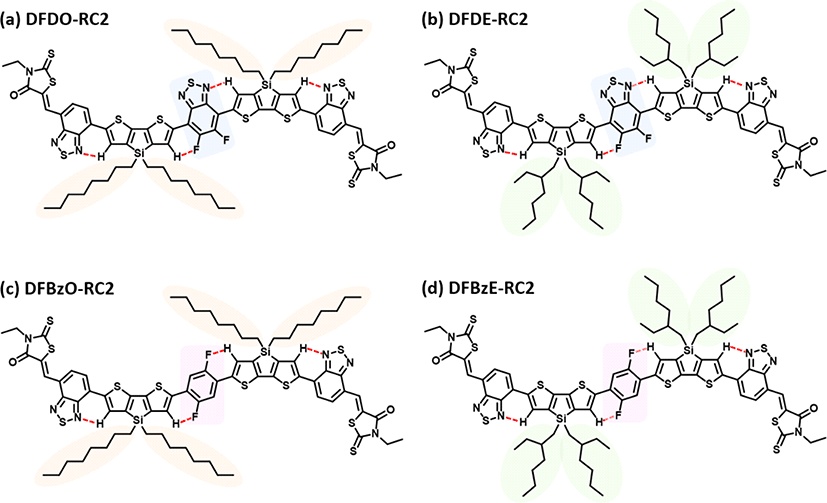
2. RESULTS AND DISCUSSION
The synthesis of NFAs (DFDO-RC2, DFDE-RC2, DFBzO-RC2, and DFBzE-RC2) are summarized in Fig. 2 and Fig. 3. The specific synthesis procedures are described in Supplementary Information. Molecules 1O, 1E, 2O, 2E, 3O, and 3E were synthesized according to the literature procedure [34]. Molecules 6O, 6E, 7O, and 7E were synthesized in a similar method. Stille coupling of 3O, 3E, 7O, and 7E with hexamethylditin afforded the 4O, 4E, 8O, and 8E, respectively. 4O, 4E, 8O, and 8E were then each coupled with 7-bromo-2,1,3-benzothiadiazole-4-carboxaldehyde to respectively yield 5O, 5E, 9O, and 9E. Subsequent Knoevenagel condensation of 5O, 5E, 9O, and 9E with 3-ethylrhodanine afforded DFDO-RC2, DFDE-RC2, DFBzO-RC2, and DFBzE-RC2 as final products. The synthesized molecules were characterized by matrix-assist laser desorption ionization time-of-flight mass spectrometry (MALDI-TOF MS) and HNMR (SI Figs. S1-S38)[1]. The synthesized NFAs are soluble in organic solvents such as toluene, chloroform, or chlorobenzene.
Density functional theory (DFT) calculations (B3LYP, 6-311G (d,p)) were carried out to investigate the electronic structures and molecular geometries of the synthesized NFAs. Fig. 4 displays the front and side views of optimized geometries of the NFAs. DFDO-RC2 and DFDE-RC2 molecules were designed to have planar structures through intramolecular non-covalent and Fig. 1(b)). DFT calculations revealed that the DFD series have highly planar backbones with dihedral angles ranging from 0.10° to 0.74° between difluorobenzothiadiazole moieties and the neighboring dithienosilole groups. The use of difluorinated benzene as a core, which can induce F⋯H and N⋯H interactions between fluorine in difluorobenzene and hydrogen in dithienosilole units, also induces the planar backbone in the DFBzO-RC2 and DFBzE-RC2 molecules (Fig. 1(c) and Fig. 1(d)). Optimized DFT calculations of the resulting DFBz series molecules showed dihedral angles ranging from 14.74° to 17.24° (Fig. 4(c) and Fig. 4(d)), which were somewhat increased compared to those of DFD molecules (Fig. 4(a) and Fig. 4(b)), indicating a slightly decreased planarity in an aromatic backbone structure. Although some minor differences were observed in the dihedral angles for DFD and DFBz series, we note that the dihedral angles exhibit values lower than 20°, so all the molecules can have an effective π-conjugation along the molecular backbone. Fig. 5 displays surface plots and energy levels of molecular HOMOs and LUMOs. The HOMO/LUMO levels were −5.323 eV/−3.556 eV for DFDO-RC2 (Fig. 5(a)) and −5.335 eV/−3.562 eV for DFDE-RC2 (Fig. 5(b)). For the DFD series, which exhibited highly planar backbone structures, electrons in both HOMOs and LUMOs were quite evenly delocalized throughout the molecules. For DFBzO-RC2 and DFBzE-RC2, the HOMO/LUMO levels were respectively calculated to be −5.376 eV/−3.461 eV and −5.391 eV/−3.467 eV (Fig. 5(c) and Fig. 5(d)), in which the HOMO levels were lower, and the LUMO levels were higher than those corresponding values of the DFD series. The HOMOs of the DFBz series are delocalized throughout the molecules, but the LUMOs are more concentrated on the electron-deficient periphery of the molecules. From the HOMO/LUMO levels obtained from DFT results, the bandgaps were calculated to be 1.767, 1.773, 1.915, and 1.924 eV for DFDO-RC2, DFDE-RC2, DFBzO-RC2, and DFBzE-RC2, respectively.
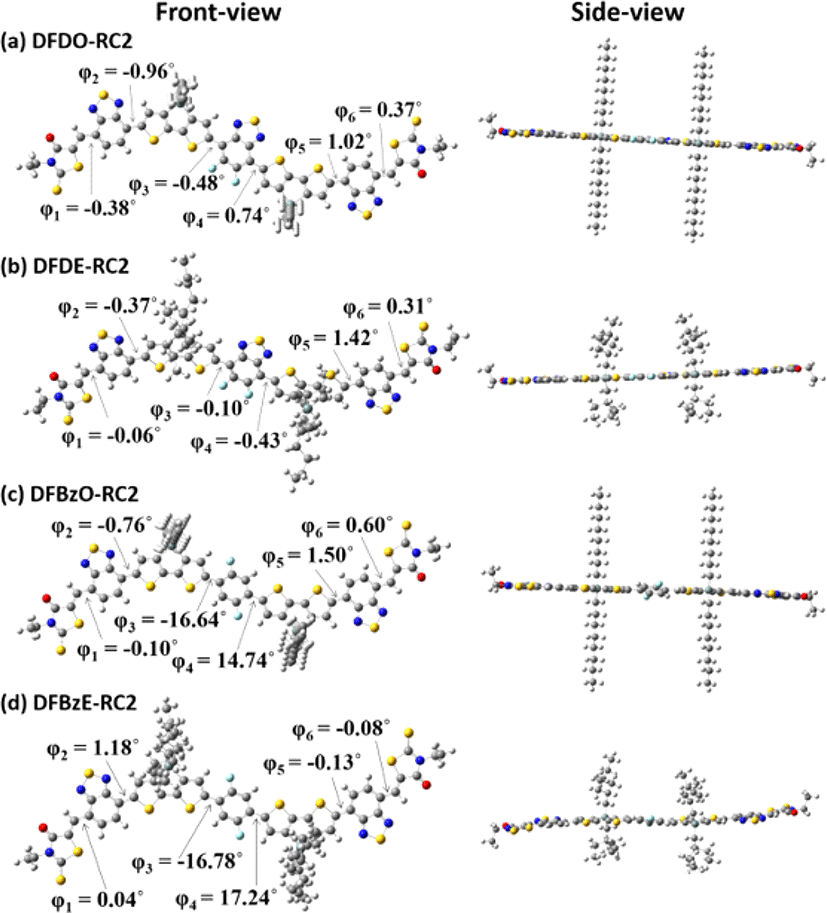
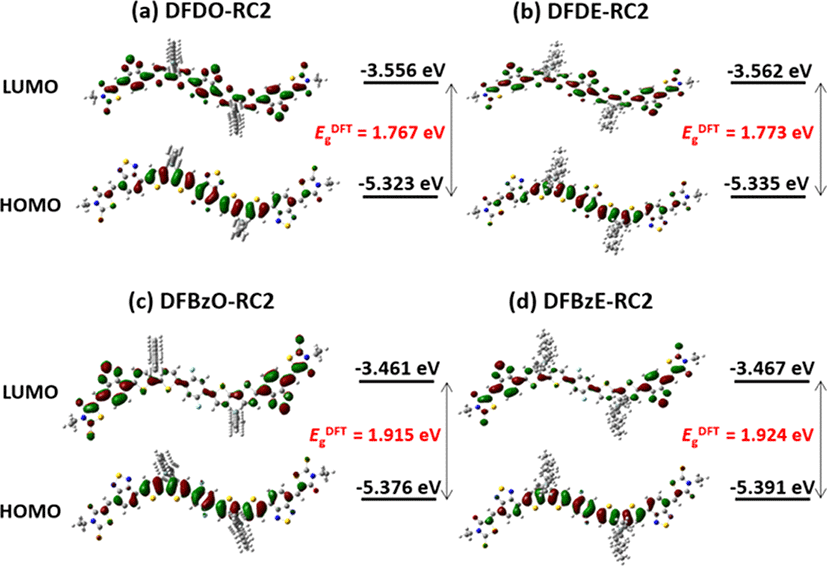
The electrochemical behaviors of the NFAs were observed through cyclic voltammetry (CV) measurements. The oxidation-reduction cycles of each NFA are drawn in Fig. 6(a)−Fig. 6(d), and an energy diagram in Fig. 6(e) summarizes the molecular energy levels determined by CV (see SI Fig. S39 for cyclic voltammogram of PTB7-Th film). The HOMO and LUMO energy levels were estimated from the onset voltages of oxidation and reduction. The HOMO/ LUMO levels of DFDO-RC2 and DFDE-RC2 were −5.20/ −3.67 eV and −5.24/−3.71 eV, respectively, which are consistent with the trend observed in the DFT results. DFBzO-RC2 and DFBzE-RC2 showed an equal HOMO level of −5.30 eV, which was lower than the HOMO levels of the DFD series molecules. This observation also agrees well with the DFT results. However, in the case of LUMO levels, CV results revealed that all four molecules exhibited similar LUMO energy values within an experimental error range. At the same time, DFT predicted that the LUMO levels of the DFBz series are higher than those of the DFD series. This observation suggests that the actual molecular backbones of the DFBz series could be more planar than the structures expected from the DFT results so that the π-conjugation between the electron-deficient side-arm units in the molecules would be more effective than expected from the DFT calculations. This fact is supported by the higher crystallinity of DFBz molecules. Importantly, the DFBz molecules showed larger HOMO-HOMO energy offsets with the PTB7-Th electron-donor polymer used in our OPV devices compared to the DFD molecules, which would facilitate effective charge separation at the interface between PTB7-Th and NFAs. All the NFAs exhibited sufficient LUMO-LUMO energy offsets with PTB7-Th, which is beneficial for the efficient separation of excitons formed in the PTB7-Th domains. We also note that there is little difference in LUMO energy levels among the four NFAs, from which we can expect similar Voc values in these NFAs contained OPV devices. From the energy levels obtained from CV, electronic bandgaps of DFDO-RC2, DFDE-RC2, DFBzO-RC2, and DFBzE-RC2 were calculated to be 1.53, 1.53, 1.60, and 1.60 eV, respectively, which is also in good agreement with the trends observed in the DFT results.
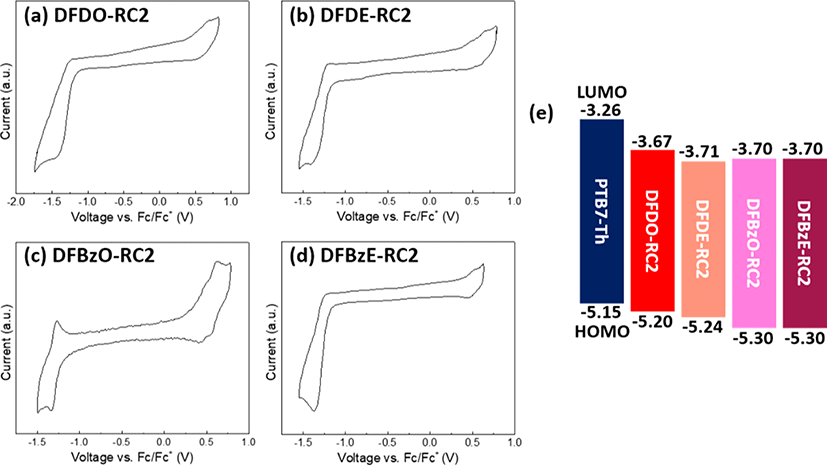
Ultraviolet (UV)-visible absorption spectroscopy measurements were carried out to investigate the optoelectronic properties of the NFAs in solution and film states. Two main absorption peaks were observed around 400 and 649 nm for the DFD series and around 414 and 614 nm for the DFBz series in chloroform solution (Fig. 7(a)). The maximum extinction coefficients of the NFAs in solution states were 1.88×104, 1.94×104, 2.51×104, and 2.54×104 M−1cm−1 for DFDO-RC2, DFDE-RC2, DFBzO-RC2, and DFBzE-RC2, respectively. These values are approximately one order of magnitude higher than that (4.90×103 M−1cm−1) of PC61BM in the solution state [37], demonstrating the potential of these NFAs to yield a greater light absorption than PC61BM. Fig. 5(b) displays molecular films of the four NFAs at room temperature (RT). Compared with the absorption in the solution state, all the NFAs displayed significant red-shifts and shoulder peaks in the near IR region, indicating strong intermolecular stacking in the film state. The maximum extinction coefficients of DFDO-RC2, DFDE-RC2, DFBzO-RC2, and DFBzE-RC2 in thin film states were 6.17×104, 6.33×104, 8.96×104, and 8.97×104 cm−1, respectively. Interestingly, the DFBz series molecules showed higher absorption characteristics in the visible light region than those of the DFD series molecules in both solution and thin film states. This feature implies that the DFBz series may yield a greater photocurrent than the DFD series. The DFD molecules showed more red-shifted absorption spectra than the DFBz molecules in both solution and thin film states (Fig. 7(a) and Fig. 7(b)). Thus, smaller optical bandgaps (: calculated from the onset wavelength in the RT films of the four NFAs) were determined in DFDO-RC2 (1.43 eV) and DFDE-RC2 (1.50 eV) than those of DFBzO-RC2 (1.54 eV) and DFBzE-RC2 (1.58 eV). The optical bandgap tendencies are following electronic bandgaps obtained from the DFT calculations and the CV measurements . NFA films were placed at four temperatures, RT, 100, 150, and 200°C for 10 min to investigate the effect of thermal annealing on molecular films. The normalized UV-visible absorption spectra of these films are displayed in Fig. 7(c)−Fig. 7(f). DFDO-RC2, DFDE-RC2, and DFBzE-RC2 films (Fig. 7(c), Fig. 7(d), Fig. 7(f)) showed a bathochromic shift and a pronounced increase of shoulder peaks in the longer wavelength region upon increasing annealing temperatures, a characteristic of J-aggregate formation. On the other hand, DFBzO-RC2 (Fig. 7(e)) interestingly exhibited a blue-shift in maximum absorption upon thermal annealing, indicating the H-aggregate formation between DFBzO-RC2 molecules [38].
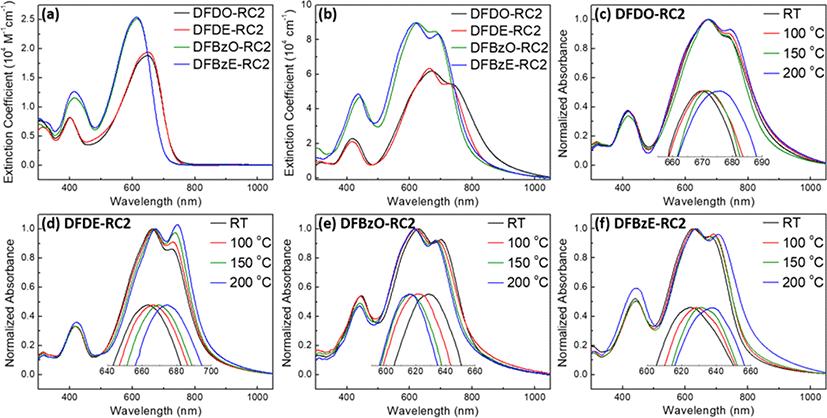
The thermal properties of small molecules were examined by differential scanning calorimetry (DSC). From the DSC curves (Fig. 8(a)∼Fig. 8(d)), melting temperatures (Tm) and crystallization temperatures (Tc) were determined, which data are summarized in Table 1. For both the DFD and DFBz series molecules, the molecules with 2-ethylhexyl side chains on dithienosilole revealed higher melting points than the corresponding molecules with octyl chains: DFDE-RC2 (Tm=320.4°C) DFDO-RC2 (Tm=288.4, 293.9°C) and DFBzE-RC2 (Tm=276.2°C) DFBzO-RC2 (Tm=259.9°C). This melting point difference can be explained by comparing coherence length (Lc) from Grazing-Incidence Wide-Angle X-ray Scattering (GIWAXS) results; DFDE-RC2 and DFBzE-RC2 revealed larger crystal sizes compared to DFDO-RC2 and DFBzO-RC2 compounds, which possibly led to greater Tm values. Notably, the molecules with linear octyl side chains exhibited more various thermal transitions than molecules with branched 2-ethylhexyl side chains. DFDO-RC2 (Fig. 8(a) showed two melting peaks at 288.4°C and 293.9°C, and DFBzO-RC2 (Fig. 8(c)) showed three crystallization temperatures of 237.7°C, 235.3°C, and 230.0°C, which suggests that liquid crystalline state might exist in these two molecules having octyl side chains [39].
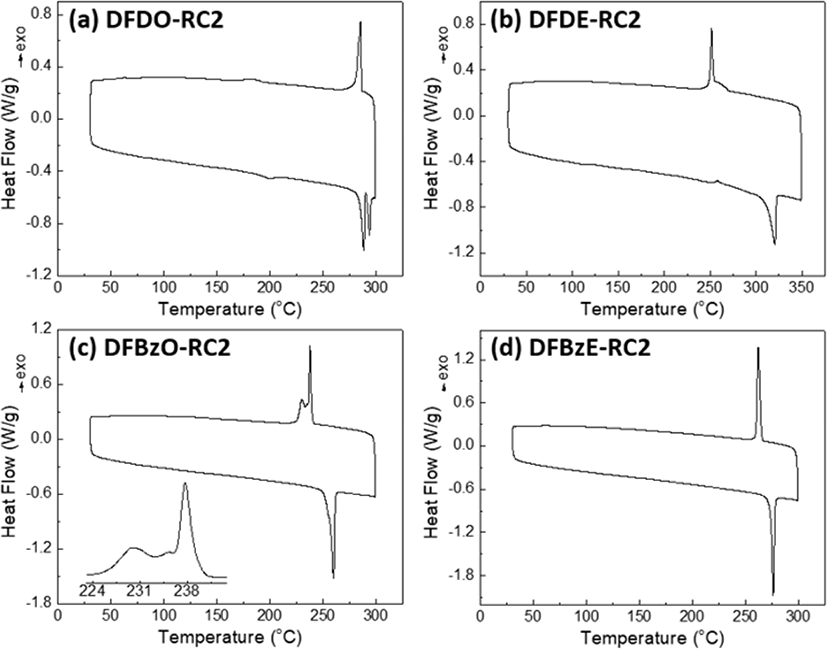
GIWAXS patterns of the molecular thin films were obtained to examine the effect of thermal annealing on the crystallinity and long-range ordering of the NFAs. The GIWAXS images of NFA films annealed at RT, 100°C, 150°C, and 200°C are shown in Fig. 9(a)−Fig. 9(d), and the GIWAXS parameters are summarized in Table 2. The line-cut profiles of films are shown in SI Fig. S40-S44. The out-of-plane profiles were drawn from the qz direction signal at qy =0.00 Å−1, and the in-plane profiles were extracted from the qy direction signal at qz =0.03 Å−1. In all the molecular films, enhanced long-range ordering of the NFAs in the qz direction was observed upon thermal annealing; that is, the peaks associated with lamellar stacking of the NFAs became stronger. As-cast DFDO-RC2 (Fig. 9(a)) and DFDE-RC2 films (Fig. 9(b)) exhibited only (100) peaks in the out-of-plane direction, and 100, 150, and 200°C – annealed films exhibited high order (200), and (300) peaks as well. For DFBzO-RC2 films (Fig. 9(c)) and DFBzE-RC2 films (Fig. 9(d)), (100), (200), and (300) peaks appeared even in the as-cast films, which means that the DFBz series molecular films are more crystalline than the DFD series molecular films and became more prominent upon thermal annealing. From the comparison of (100) peaks of the NFAs, it is found that the backbone structure of the DFBz series facilitates molecular packing better; the (100) peak of DFDO-RC2 appeared at qz=0.396 Å−1 (d=15.884 Å), and the (100) peak of DFBzO-RC2 showed at qz=0.426 Å−1 (d=14.760 Å), which indicates that octyl chains in DFBzO-RC2 are more closely interdigitated, thus showing more closely packed lamellar structure compared to DFDO-RC2. A similar feature was found between DFDE-RC2 and DFBzE-RC2. These results suggest that the molecular backbone of the DFBz series in film state could be more planar than the structure observed in the DFT results. The (200) and (300) peaks were more prominent in 100, 150, and 200°C films of DFDO-RC2 than in DFDE-RC2 films (Fig. 9(a) and Fig. 9(b)). For all the NFA films, (010) peaks were observed in the in-plane direction, indicating that all the NFAs tend to adopt an edge-on orientation mainly. In as-cast molecular films, the π-π stacking peak position and the corresponding d spacing values of each molecule are as follows: DFDO-RC2 (qy = 1.775 Å−1 (d=3.539 Å)), DFDE-RC2 (qy =1.781 Å−1 (d=3.527 Å)), DFBzO-RC2 (qy =1.774 Å−1 (d =3.543 Å)), and DFBzE-RC2 (qy =1.747 Å−1 (d = 3.598 Å)). DFDO-RC2 and DFDE-RC2 had slightly more closely packed π-π stacking structures than DFBzO-RC2 and DFBzE-RC2. The closed π-π stacking may be associated with the fact that the maximum absorption peak in the UV is more red-shifted in the DFD series than the DFBz series when going from the solution state to the film state (Fig. 5(a) and Fig. 5(b)). These facts may be attributed to the characteristic of the more heteroatom-contained planar difluorobenzothiadiazole moiety, which gives rise to strong intramolecular interactions with planar structure compared to a simpler difluorobenzene moiety. According to the coherence length values in the qz direction, the relatively larger crystal sizes of DFDE-RC2 and DFBzE-RC2 were observed compared to DFDO-RC2 and DFBzO-RC2. This fact is in accordance with UV-visible absorption spectral features (Fig. 7(b)); molecules with 2-ethylhexyl as side chains showed more prominent absorption changes upon thermal annealing than the corresponding molecules with octyl side chains. This result is because the shorter alkyl chain length of 2-ethylhexyl chains may have enhanced the molecular stacking in DFDE-RC2 and DFBzE-RC2 molecules [40].
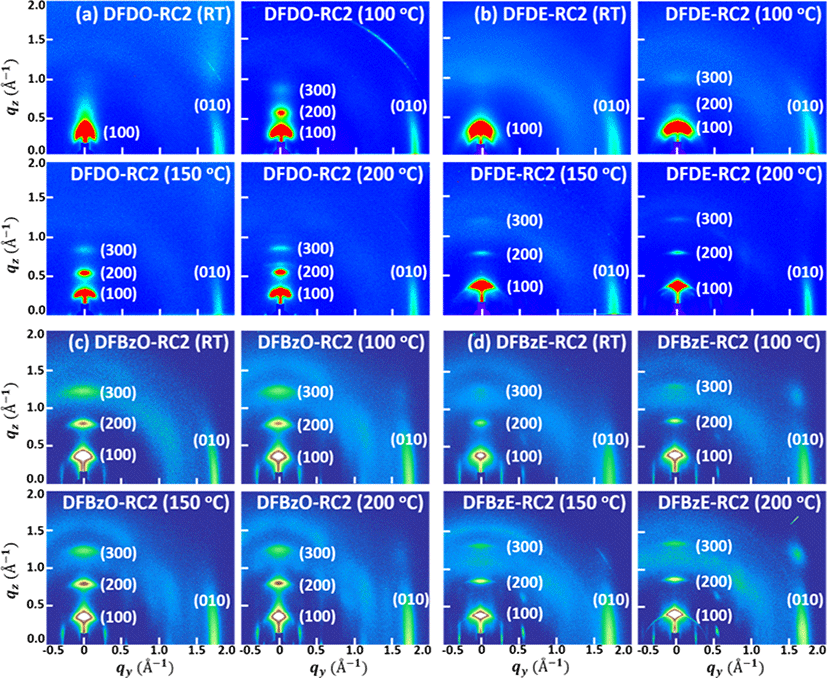
OPV devices were fabricated with the inverted type architecture: glass/ITO/ZnO/PEIE/PTB7-Th:NFA/V2O5/ Ag. J-V curves and external quantum efficiency (EQE) spectra of optimized OPV devices are shown in Fig. 10(a) and Fig. 10(b), and their photovoltaic performance parameters are summarized in Table 3. PTB7-Th: DFBz devices yielded outstanding open-circuit voltages of over 1 V, while PTB7-Th:DFDO-RC2 and PTB7-Th:DFDE-RC2 devices exhibited relatively lower Voc values of 0.88 V and 0.78 V, respectively. Higher Voc values were obtained in PTB7-Th:DFBz devices due to the relatively low energy loss (Eloss) of 0.51 eV in the PTB7-Th:DFBzO-RC2 and PTB7-Th:DFBzE-RC2 devices. This low Eloss value is comparable to that of inorganic solar cells [41-42], and also is among the lowest value achieved in NFA-based polymer solar cells reported so far [13],[43-47]. The energy loss was calculated from the equation where Eg corresponds to the optical bandgap of the NFAs [13,48-49]. A higher short-circuit current (Jsc) of 4.39 mA/cm2 was obtained from PTB7-Th :DFDE-RC2 devices, compared with 0.58 mA/cm2 for PTB7-Th:DFDO-RC2 devices. The high Jsc can be explained by the fact that DFDE-RC2 has a greater HOMO-HOMO energy offset with PTB7-Th and a finer surface morphology than DFDO-RC2 (see below the morphology study). Relative to the DFD series, higher Jsc values (2.38 mA/cm2 for PTB7-Th: DFBzO-RC2 devices and 10.53 mA/cm2 for PTB7-Th: DFBzE-RC2 devices) were obtained in DFBz series. Higher photocurrent density in the DFBz series can be accounted for by the larger HOMO-HOMO energy offset with PTB7-Th than the DFD series. In addition, the DFBz series has higher extinction coefficients than the DFD series. Among the DFBz series, PTB7-Th: DFBzO-RC2 blend film revealed a rough morphology with large aggregates, while PTB7-Th:DFBz-RC2 blend film had the ideal morphology among the four PTB7-Th:NFA blend films which can be accounted for the highest Jsc and FF values for PTB7-Th:DFBz-RC2 devices (see below the morphology study). As a result, the highest efficiency of 4.85% was obtained from the PTB7-Th:DFBzE-RC2 device, where the three photovoltaic performance parameters, Voc, Jsc, and FF were the highest. EQE measurements were performed to confirm the Jscs of the devices. The integrated current densities were calculated to be 0.57 (PTB7-Th:DFDO-RC2), 4.61 (PTB7-Th:DFDE-RC2), 1.92 (PTB7-Th: DFBzO-RC2), and 9.31 mA/cm2 (PTB7-Th:DFBzE-RC2), which were in good agreement with the measured Jsc values.
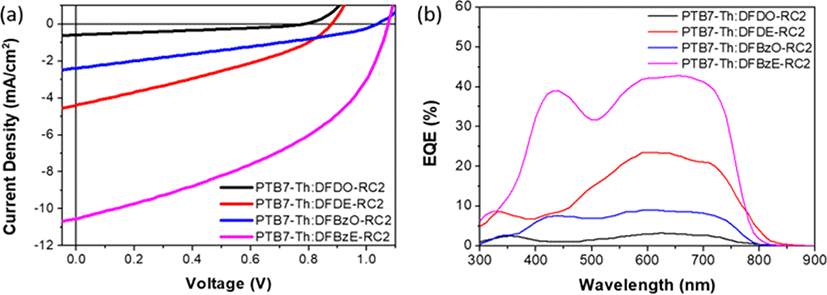
Transmission electron microscopy (TEM) images of the PTB7-Th:NFA blend films were obtained to investigate the fibril structures in the blend films (Fig. 11(a) −Fig. 11(d)). If the domain size is too large, geminal recombination occurs before the exciton reaches the electron-donor/electron-acceptor interface, and if the domain size is too small, the chance of bimolecular recombination increases. Therefore, phase separation at an appropriate length scale is important for the parameters of Jsc and FF. Large branch-like aggregations were observed in PTB7-Th:DFBzO-RC2 film (Fig. 11(c)), whereas the other three blend films showed phase separation at the nanoscale (Fig. 11(a), Fig. 11(b), Fig. 11(d)). The large aggregation resulted in the low Jsc and FF obtained from the PTB7-Th: DFBzO-RC2 device.
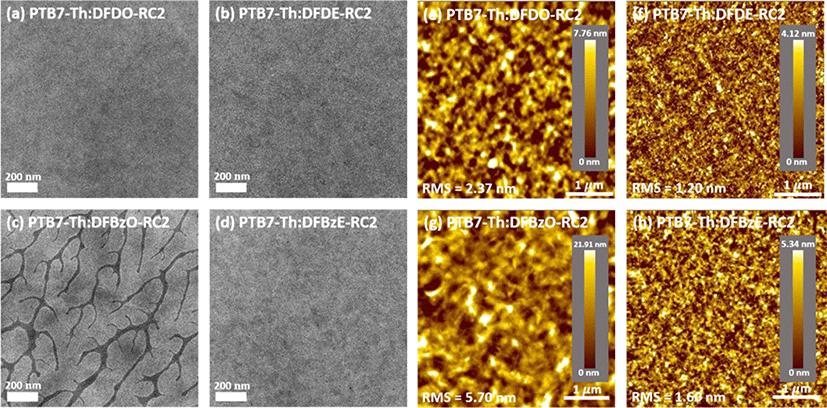
In addition, surface morphologies of the PTB7-Th:NFA blend films were examined using atomic force microscopy (AFM) under non-contact mode (Fig. 11(e)−Fig. 11(h)). The root mean square (RMS) values of PTB7-Th:DFDO-RC2, PTB7-Th:DFDE-RC2, PTB7-Th: DFBzO-RC2, and PTB7-Th:DFBzE-RC2 films were 2.37, 1.20, 5.70, and 1.60 nm, respectively. PTB7-Th: DFBzO-RC2 exhibited large-sized grains (Fig. 11(g)), which agrees well with images obtained from TEM. Among the three blend films that showed nanosized fibrillar structure in TEM, relatively rough morphology was observed in PTB7-Th:DFDO-RC2 blend film (Fig. 9(e)). It appears that the electron-donor and electron-acceptor domains are clustered together, in which recombination occurs before the resulting electrons and holes generated in each domain reach the electrodes. PTB7-Th:DFDE-RC2 and PTB7-Th:DFBzE-RC2 blend films showed relatively smooth surface morphology. PTB7-Th:DFDE-RC2 exhibited a too fine surface feature (Fig. 11(f)), which induces bimolecular recombination, while PTB7-Th:DFBzE-RC2 exhibited a moderately smooth surface with nanoscale domains (Fig. 11(d) and Fig. 11(h)). Therefore, the highest Jsc and FF were obtained in the PTB7-Th:DFBzE-RC2 device containing the ideal morphology among the four blend films.
GIWAXS measurements were conducted on the PTB7-Th:NFA blend films to investigate the formation of pure electron-donor and electron-acceptor domains in the blend films. The images of PTB7-Th:DFDO-RC2, PTB7-Th:DFDE-RC2, PTB7-Th:DFBzO-RC2, and PTB7-Th:DFBzE-RC2 blend films are shown in Fig. 12(a)−Fig. 12(d), and the GIWAXS parameters of the four blend films are summarized in Table 4. The line-cut profiles in the qz and qy directions of the blend films are shown in SI Figs. S45, S46. The blend films were compared with their corresponding molecular films. All the blend films showed more reduced crystallinity, indicating that less ordered phases were formed by blending NFA molecules with PTB7-Th, compared to their molecule-only film counterparts. Nevertheless, the crystalline features of NFAs were maintained to some degree in PTB7-Th:DFDO-RC2 (Fig. 12(a)) and PTB7-Th:DFBzO-RC2 (Fig. 12(c)) films. In PTB7-Th:DFDO-RC2 film, (100) peak coming from DFDO-RC2 and PTB7-Th (see SI Fig. S47 for GIWAXS data of PTB7-Th film) were observed at qz =0.316 Å−1 (d=19.859 Å), and (200) and (300) peaks coming from DFDO-RC2 appeared at qz =0.624 Å−1 and qz =0.931 Å-1, respectively. The π-π stacking structure in PTB7-Th:DFDO-RC2 film was observed at qy =1.770 Å−1 (d =3.549 Å). The characteristic (010) peak of PTB7-Th (qz =1.591 Å−1, d =3.948 Å) was not well observed in PTB7-Th:DFDO-RC2 film, indicating that PTB7-Th and DFDO-RC2 are mixed. In PTB7-Th:DFBzO-RC2 film, (100) peak coming from DFBzO-RC2 and PTB7-Th were observed at qz=0.422 Å−1 (d=14.883 Å), and very strong (200) and (300) peaks coming from DFBzO-RC2 respectively appeared at qz=0.840 Å−1 and qz=1.242 Å−1. Also, the π-π stacking structure of DFBzO-RC2 was observed at qy=1.766 Å−1 (d=3.558 Å), and a peak indicating the face-on domains of PTB7-Th was observed at qz=1.717 Å−1 (d=3.660 Å). That is, in PTB7-Th:DFBzO-RC2 blend film, the phase separation between PTB7-Th and DFBzO-RC2 was more prominent compared to PTB7-Th:DFDO-RC2 blend film. PTB7-Th:DFDE-RC2 and PTB7-Th:DFBzE-RC2 blend films showed a more amorphous-like feature relative to PTB7-Th: DFDO-RC2 and PTB7-Th:DFBzO-RC2 blend films. In PTB7-Th:DFDE-RC2 film, (100) peak coming from DFDE-RC2 and PTB7-Th appeared at qz=0.333 Å−1 (d= 18.870 Å), π-π interaction peak was observed at qz= 1.667 Å−1 (d=3.770 Å). This observation agrees with the too well-mixed phase observed in the AFM image of PTB7-Th:DFDE-RC2 film (Fig. 11(f)). In PTB7-Th:DFBzE-RC2, the (100) peak from DFBzE-RC2 and PTB7-Th appeared at qz=0.419 Å−1 (d= 14.981 Å) and π-π interaction peak was observed at qz=1.730 Å−1 (d=3.631 Å). Additionally, peaks observed at qz=1.300 Å−1 (d=4.833 Å) and qy=1.724 Å−1 (d=3.644 Å) can be assigned to the crystal packing of DFBzE-RC2 molecules. Therefore, PTB7-Th:DFBzE-RC2 film exhibited a moderately-mixed phase, while the electron-donor and electron-acceptor materials formed their own domains. Although the degree of phase separation in each blend film differs, the results from blend film AFM, TEM, and GIWAXS suggest that a certain amount of phase separation exists in all the blend films.
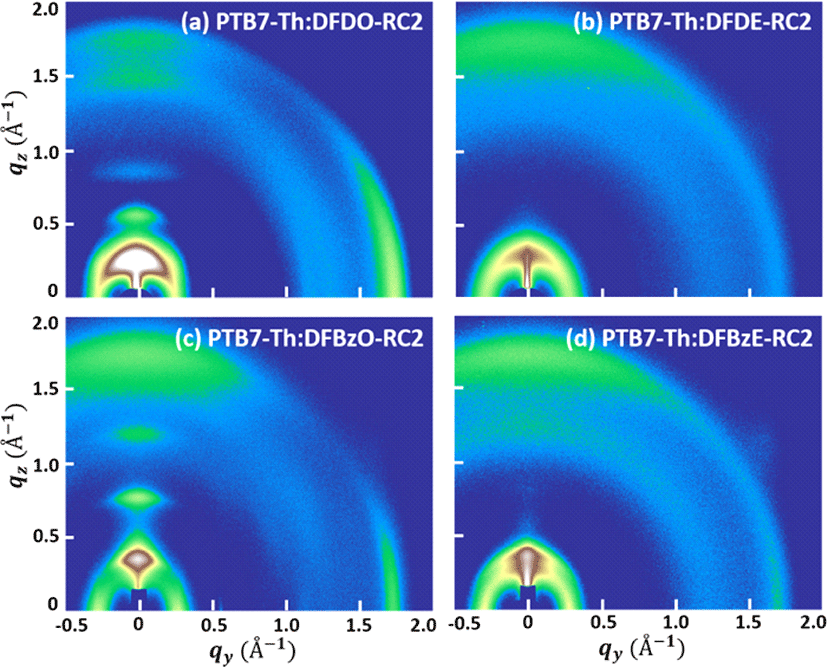
The hole and electron mobilities (μh and μe) of the PTB7-Th:NFA blend films were calculated using the space charge-limited current (SCLC) model. Current density-voltage curves of hole-only and electron-only devices are shown in Fig. 13(a) and Fig. 13(b), and the obtained carrier mobilities are summarized in Table 5. Among the four kinds of devices, PTB7-Th: DFBzO-RC2 device showed the highest μh and μe of 3.19×10−4 cm2 V−1 s−1 and 7.11×10−5 cm2 V−1 s−1, respectively. The high carrier mobilities observed in PTB7-Th:DFBzO-RC2 device suggests that branch-like aggregates observed in the TEM data of PTB7-Th: DFBzO-RC2 blend film (Fig. 9(c)) may have acted as a good pathway for charge carrier transport. However, the rough morphology and too big phase separation of PTB7-Th:DFBzO-RC2 film was detrimental to device performance. Comparing the PTB7-Th: DFDO-RC2 device (μh=6.20×10−4 cm2 V−1 s−1 and μe= 1.84×10−5 cm2 V−1 s−1) with the PTB7-Th:DFDE-RC2 device (μh= 5.34×10−4 cm2 V−1 s−1 and μe=4.98× 10−6 cm2 V−1 s−1), both μh and μe were higher in the PTB7-Th: DFDO-RC2 device, but a higher PCE was observed in PTB7-Th:DFDE-RC2 device. This is because the PTB7-Th: DFDE-RC2 device has better surface morphology and a larger HOMO-HOMO energy offset between PTB7-Th and DFDE-RC2, affecting photovoltaic performance more significantly. The PTB7 -Th:DFBzE-RC2 device (μh=7.19×10−5 cm2 V−1 s−1 and μe=4.43×10−5 cm2 V−1 s−1), which showed the highest PCE, revealed the most balanced hole-electron mobility ratio (μh/μe) of near 1. Balanced carrier transport improves charge collection at each electrode with reduced bimolecular recombination.
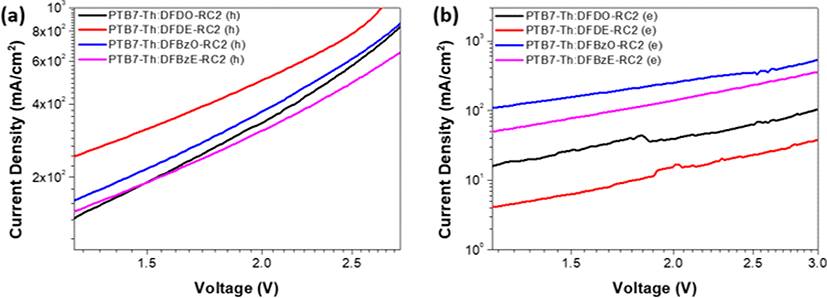
3. CONCLUSION
In conclusion, we have designed and synthesized four new small electron-acceptor molecules DFDO-RC2, DFDE-RC2, DFBzO-RC2, and DFBzE-RC2. DFBzO-RC2 and DFBzE-RC2 exhibited down-shifted HOMO levels compared to DFDO-RC2 and DFDE-RC2, which led to greater HOMO-HOMO energy offsets with PTB7-Th and thus induced more efficient exciton separation at the interface. Moreover, the DFBz series molecules showed higher extinction coefficients in the visible region than the DFD molecules, contributing to the Jsc increase. Compared to the relatively more well-mixed phase observed in the DFD series blend films, the DFBz series blend films demonstrated a higher degree of phase separation with increased crystallinity in the blend films. PTB7-Th:DFBz-RC2 blend film revealed the most optimal morphology among the four PTB7-Th:NFA blend films. Therefore, with a simple change to DFBz in the core structure, the PTB7-Th:DFBzE-RC2 device exhibited a significant increase in PCE (4.85%), with a high Voc of 1.07 eV and a very low Eloss of 0.51 eV, while the DFD series devices obtained low power conversion efficiencies (1.30%). Overall, this work demonstrates how the simple change of backbone structure influences the molecular energy level, molecular crystallinity, and morphology. Also, it shows how alkyl chain type affects the molecular interaction in the molecular-only and PTB7-Th:NFA blend films. These understandings provide insights into developing future high-performance non-fullerene acceptor molecules and improving OPV performance.