1. INTRODUCTION
Mechanically deformable, and thus soft, electronic devices can be broadly categorized into flexible electronics, which can be significantly bent, and stretchable electronics, a more advanced concept that allows for stretching. Soft electronics overcome the physical and mechanical limitations of traditional rigid electronic devices and enable new functionalities that were previously impossible. As a result, they have been a focus of intensive research and development since the 2000s [1-10]. Key application areas for soft electronics include bendable or stretchable flexible displays, digital healthcare applications for collecting biometric data through invasive or non-invasive methods, and the Internet of Things, which gathers information from the surrounding environment or objects and utilizes it for user interaction. Among these, digital healthcare is particularly notable. It allows real-time monitoring of users' health conditions, enabling the prevention of emergencies and the implementation of appropriate measures when needed. These capabilities offer significant benefits to daily life. Additionally, driven by the explosive demand for health-related technologies during the pandemic era, the market for digital healthcare has been experiencing rapid growth [11].
The definition of digital healthcare is indeed broad, and as it involves the convergence of diverse technologies from various fields to create new domains, it is not easy to provide a single, definitive explanation of what “digital healthcare” is. However, digital healthcare technologies based on soft electronic devices primarily aim to perform the following four functions (Fig. 1). First is to collect biometric signals, which involves inserting or attaching soft electronic devices to the desired body organs or tissues to acquire high-quality biometric signals with high accuracy. The second is processing of acquired biometric signals - once collected, the biometric signals need to be converted from analog to digital form, stored, and undergo post-processing, such as amplification, to improve their readability for the user. Third, the processed biometric data should be transmitted to other electronic devices (such as smartphones or computers) so that users can access the information. And lastly, based on the acquired biometric data, appropriate feedback should be delivered as needed (e.g., drug delivery from embedded systems), ensuring precise user operation and control.
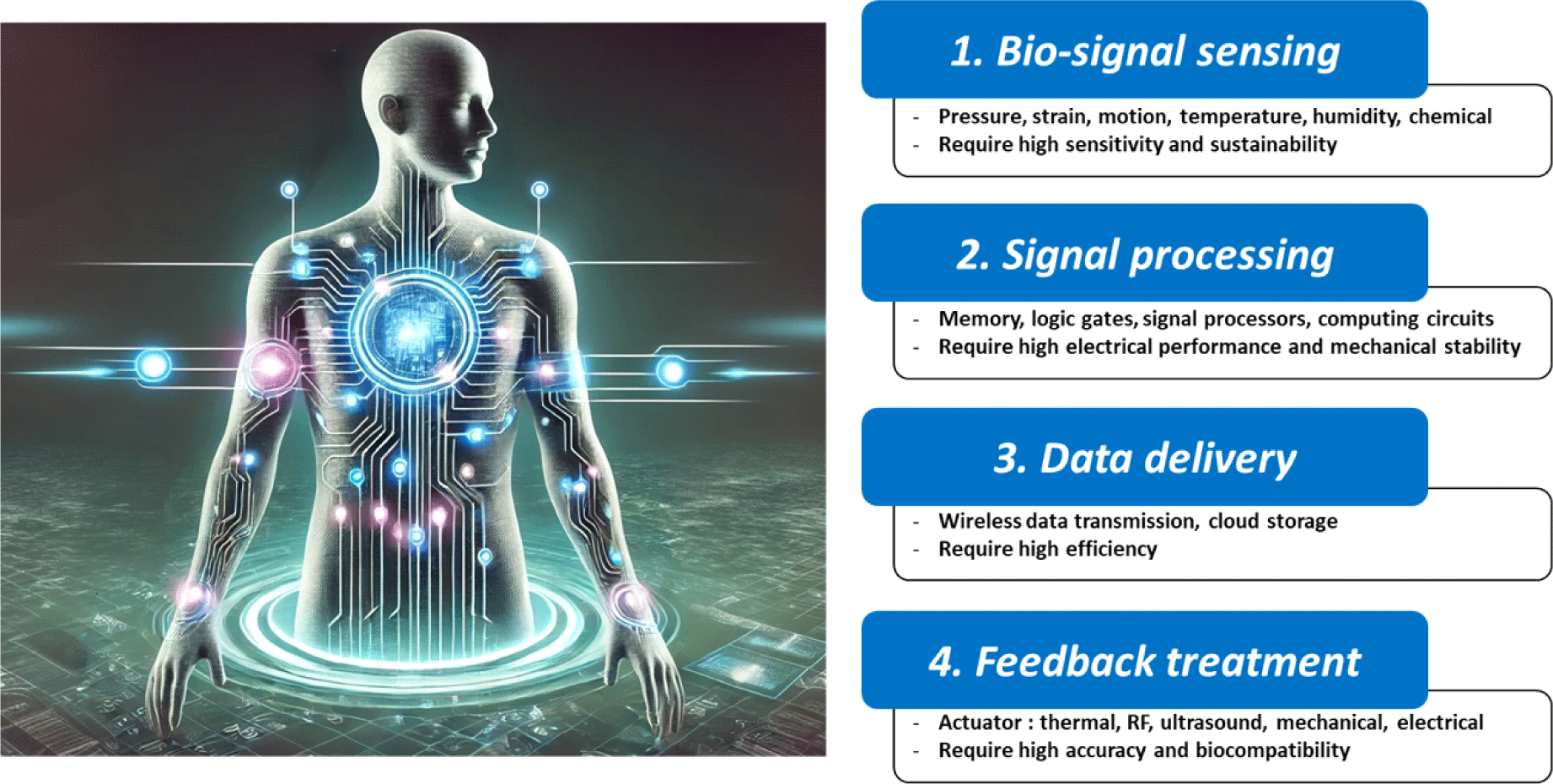
Digital healthcare technologies based on flexible electronic devices aim to establish a closed-loop system capable of achieving both diagnostic objectives—accurately collecting the user's biometric signals—and therapeutic objectives—providing appropriate feedback based on the collected data. Although research on flexible electronic device technologies, essential for performing the four aforementioned functions, has been actively conducted for about a decade, most of it remains at an experimental stage, with various challenges yet to be overcome.
One of the most significant technical challenges in the fabrication of soft electronic devices for digital healthcare lies in matching the physical properties of the devices with those of the body’s organs and tissues where they will be inserted or attached [12-14]. This is because the human body is inherently soft, capable of stretching, contracting, and undergoing physical deformation based on its specific structures. If an electronic device with mismatched physical properties is inserted or attached, the mechanical mismatch at the interface between the body tissue and the electronic device can lead to several undesired issues. These include not only rapid device failure but also inflammation and other unintended adverse effects on the attached or implanted area.
In particular, for biometric signal collection technologies, if the electronic device does not achieve conformal contact with the area where the signals are being collected, the quality of the obtained biometric signals deteriorates significantly [15,16]. Additionally, the daily movements of the human body can disrupt the contact between the device and body tissue, potentially damaging both the device and the tissue. To ensure conformal contact between the device and the tissue, one approach involved reducing the device's thickness to within a few tens of micrometers. This increased the device's flexibility, allowing it to conform completely to tissues with complex surface structures, such as the brain. Consequently, the quality of the collected biometric signals significantly improved compared to thicker devices [15]. Another approach involved attaching nano-pillar structures on the underside of the device, inspired by the biological tissue of beetles. These structures maximized the contact area between the device and the tissue, thereby enhancing the quality of the collected signals [16].
While both methods are highly effective, they have notable drawbacks. When a device becomes too thin, it becomes difficult to handle, and its durability decreases dramatically. Furthermore, many body tissues undergo dynamic movements, such as contraction and expansion, which these devices cannot adequately accommodate. As a result, a more fundamental solution is needed. One such approach is to match the mechanical properties of the device with those of the body tissue. Therefore, to make electronic devices soft and adjust their physical properties, stretchable electronic device technology has emerged as a solution. This review paper aims to present recent research trends in stretchable electronic device technology with a focus on the intrinsically stretchable devices built from intrinsically stretchable functional materials, and explore examples of its application in bioelectronic devices.
2. MATERIAL STRATEGIES FOR DEVELOPMENT OF SOFT BIOELECTRONICS
One of the most critical considerations in the development of soft bioelectronic devices is ensuring that their electrical performance remains stable under physical or mechanical deformation. To achieve this, the materials comprising the electronic device must effectively dissipate the stress caused by such deformation. A widely researched approach involves making the materials used in electronic devices extremely thin (on the order of a few micrometers or less) to enhance their flexibility. Another method involves adopting structural device designs that can effectively dissipate strain via structural deformation. These structural designs include pre-strained buckling structures, island-bridge structures connected using serpentine interconnects, and kirigami structures (Fig. 2) [10,17].
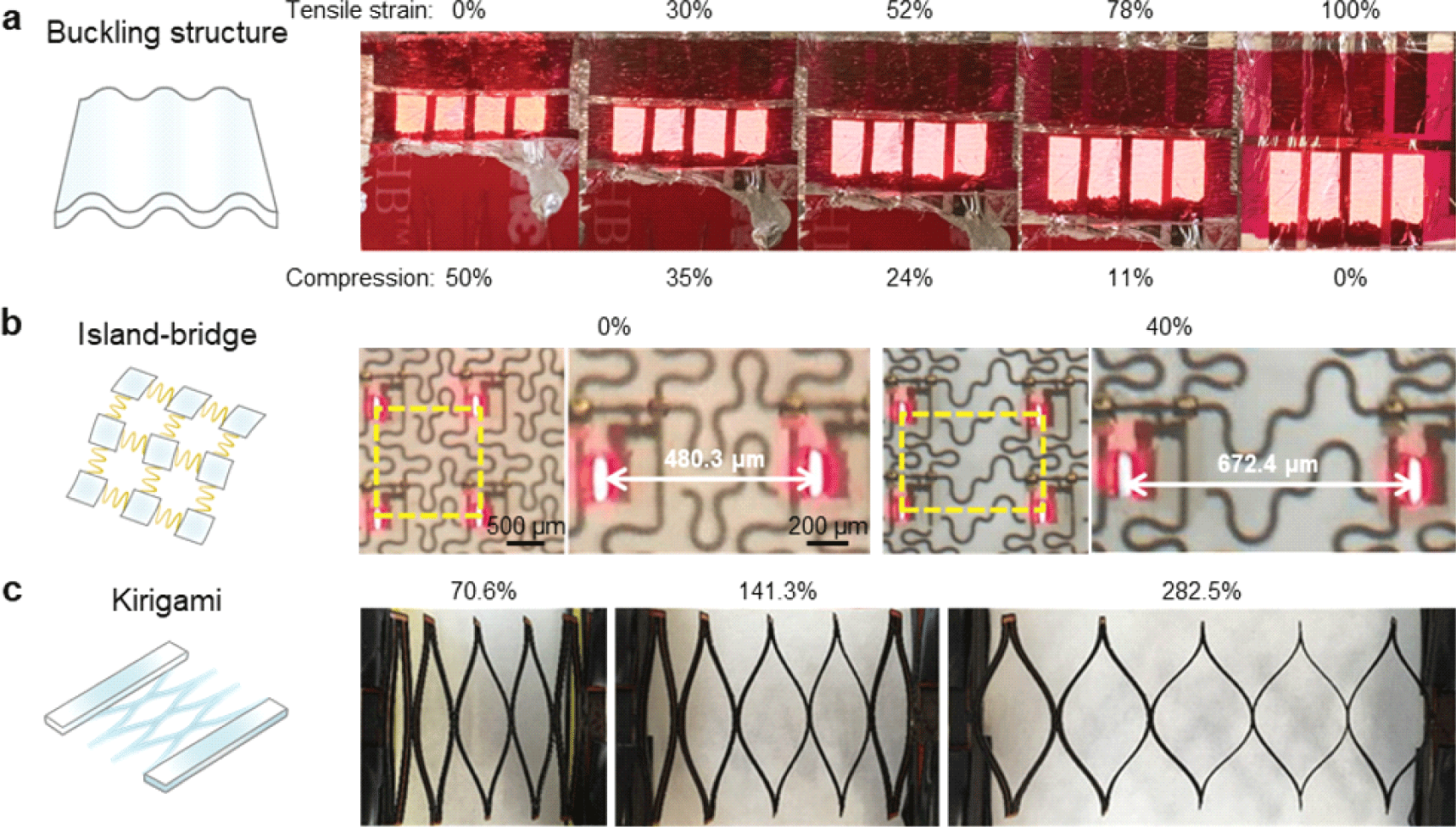
In 2011, Professor Rogers' research team from the Department of Materials Science and Engineering at Northwestern University (then affiliated with the University of Illinois at Urbana-Champaign) first introduced skin-attachable silicon electronic devices using filamentary interconnects [18]. Since then, many researchers have adopted similar approaches, modifying the geometrical structure of electronic devices to develop stretchable electronics, with a focus on applications in medical devices. For example, in 2014, Son et al. [19] developed various electronic components—including sensors, memory devices, and heaters—capable of stretching by up to 25%, mimicking the flexibility of human skin. They further incorporated nanoparticles to create skin-attachable electronic patches for drug delivery and other therapeutic applications (Fig. 3). The research team designed a system where the stretchable sensor could measure movement disorder patterns, store and analyze the data in memory devices to detect signs of disease, and use the heater to ensure efficient drug delivery from nanoparticles to the skin.
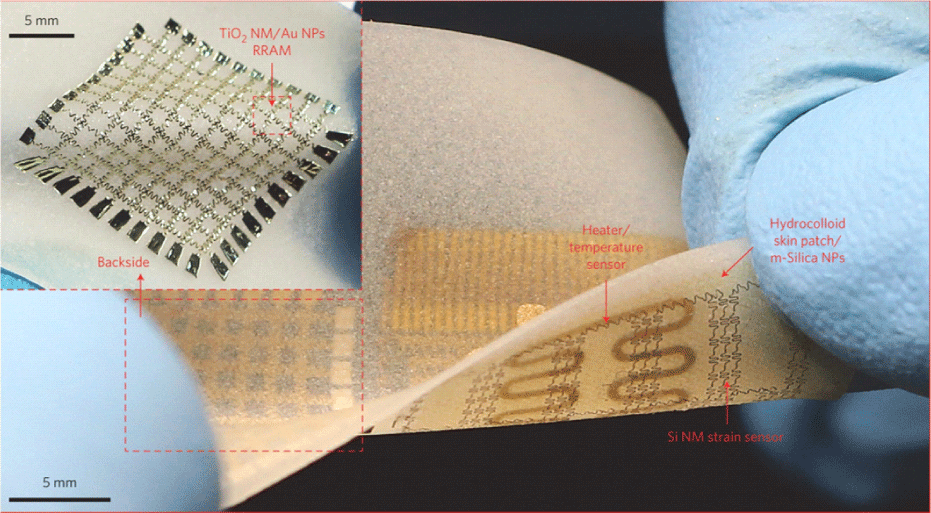
In 2015, Choi et al. [20] developed a skin-attachable heater device capable of performing thermal therapy when attached to joint areas by creating a composite of silver nanowires and an elastomer. The research team synthesized a highly conductive and uniformly high-quality composite through a ligand exchange reaction between the silver nanowires and the elastomer. To minimize discomfort when the device was attached to joint areas, they designed the device in a serpentine filamentary structure. The device demonstrated remarkable durability, showing almost no change in conductivity even when stretched to 100% strain. When a voltage of 0.45 V was applied, it generated heat of approximately 40?, and when 0.75 V was applied, it reached about 60?. The device maintained its performance even when stretched up to 60%. Leveraging these characteristics, the research team developed a heater device that could wrap around the wrist, effectively delivering heat for thermal therapy regardless of the diverse joint movements of the wrist (Fig. 4).
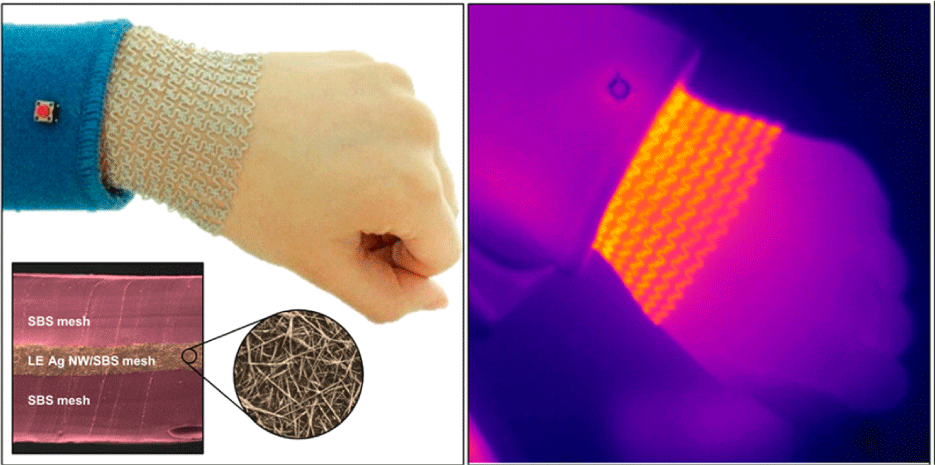
Song et al. [21], inspired by the structure of insect eyes, developed a wide-angle digital camera lens technology capable of three-dimensional imaging when attached to curved surfaces. Traditional planar lenses made from rigid inorganic materials often suffer from light distortion at the edges. However, by using convex lenses similar to insect eyes, objects can be viewed directly from all angles. The research team addressed this concept by arranging individual lenses in a curved configuration on a surface using serpentine-shaped filamentary interconnects. They demonstrated that this setup enabled the device to operate effectively, achieving a clear three-dimensional field of view (Fig. 5).
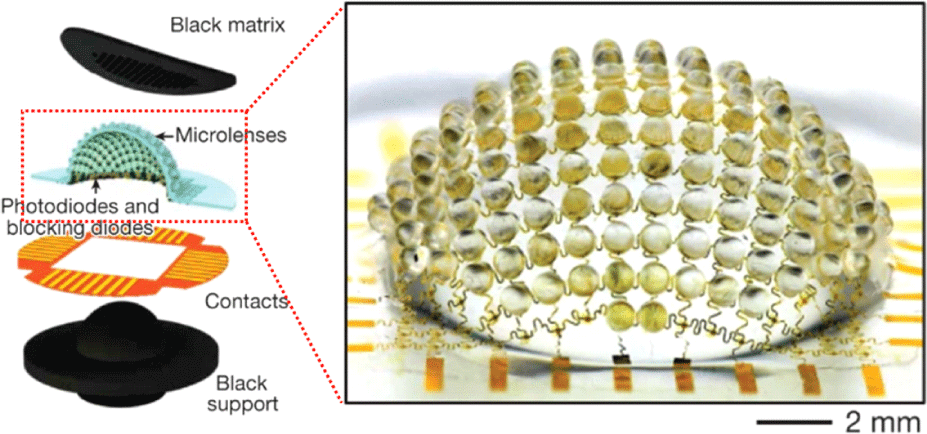
The method of adding stretchability to electronic devices by using geometrically stretchable structures has several advantages. Notably, it allows the continued use of materials already employed in conventional electronic devices, ensuring the devices' electrical performance, and it is compatible with existing semiconductor equipment and processes. However, this approach also has significant drawbacks. Repeated external stress can cause material fatigue, leading to device failure or breakage. Additionally, the need to include interconnects to dissipate stress limits the design of complex circuits and prevents the increase of device density and integration. As a result, recent research has focused on replacing the materials used in electronic devices with intrinsically soft materials [22-27]. These materials exhibit superior physical and mechanical properties compared to traditional materials but tend to have lower electrical and chemical performance. Consequently, ongoing research aims to improve their electrical and chemical performance to make them more suitable for advanced applications.
The three primary materials that constitute electronic devices are conductors, semiconductors, and dielectrics. To develop intrinsically soft bioelectronics, all materials used in electronic devices must inherently possess stretchability. Among these three, conductors stand out because they can independently perform certain electronic functions (such as in heater devices), making them highly versatile. As a result, research into intrinsically soft conductors has been more actively pursued compared to the other two materials [28-31]. The most common method for fabricating intrinsically soft conductors involves creating composites by mixing various structurally conductive nanomaterials or polymer materials with an elastomer (Fig. 6). This approach can also be applied to the fabrication of intrinsically soft semiconductors. By adjusting the ratio of the nanomaterials/polymers to the elastomer, the desired electrical and mechanical properties of the composite can be achieved. Generally, as the proportion of nanomaterials/polymers increases, the electrical performance of the composite improves, but its mechanical flexibility decreases, reflecting an inverse relationship.
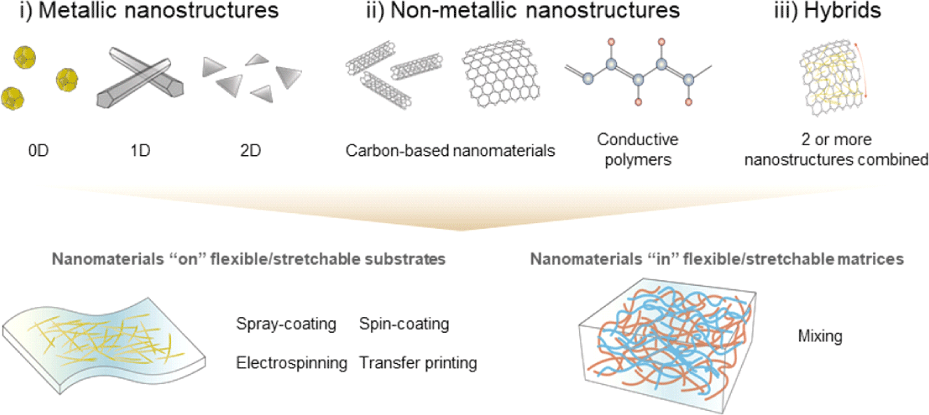
Seo et al. [32] developed an intrinsically soft conductor material with excellent biocompatibility and high mechanical and electrical durability by combining gold nanoshell-coated silver microparticles with a polymer material possessing self-healing properties. The conductive particles, composed of gold nanoshell-silver microparticles, exhibited a dynamic rearrangement property under mechanical stress, allowing the material to spontaneously restore its conductivity (Fig. 7). Using this material, the research team developed fatigue-resistant peripheral neuroprosthetics. Peripheral nerves are mostly located between muscle tissues, so implanted neuroprosthetic devices are subjected to repeated and intense mechanical stress due to muscle contraction and relaxation. This stress often leads to material fatigue and degradation of electrical performance. However, the team demonstrated that their neuroprosthetic device could stably collect sensory signals for five weeks after implantation and spontaneously recover functionality even after degradation caused by mechanical deformation.
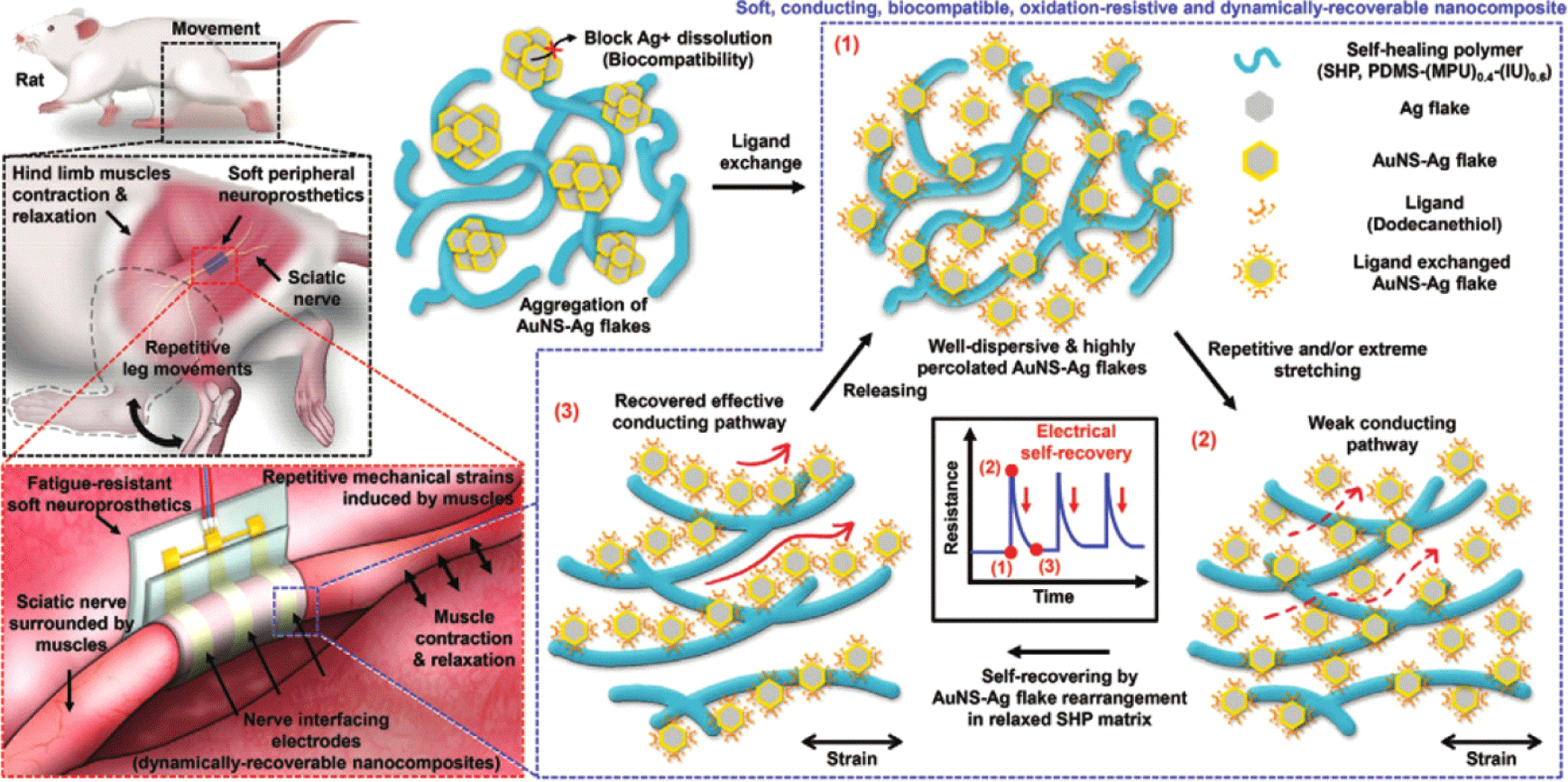
Roh et al. [33] developed a transparent, intrinsically soft conductor and a device capable of detecting even the slightest stress when attached to the skin. They achieved this by depositing a sandwich structure of the conductive polymer polyurethane-poly(3,4-ethylenedioxythiophene) polystyrenesulfonate (PU-PEDOT:PSS) and carbon nanotubes (CNT) onto an elastomer, polydimethylsiloxane (PDMS), in an extremely thin layer. The study demonstrated that the optical transparency and sensitivity of the developed device could be adjusted by controlling the thickness of the PU-PEDOT: PSS/CNT/PU-PEDOT:PSS sandwich structure and the CNT content. As a result, they successfully fabricated a sensor device that could stretch up to 100% while maintaining 62% transparency. The research team further showed that when the device was attached to facial skin, it could detect subtle muscle movements corresponding to emotional changes (Fig. 8).
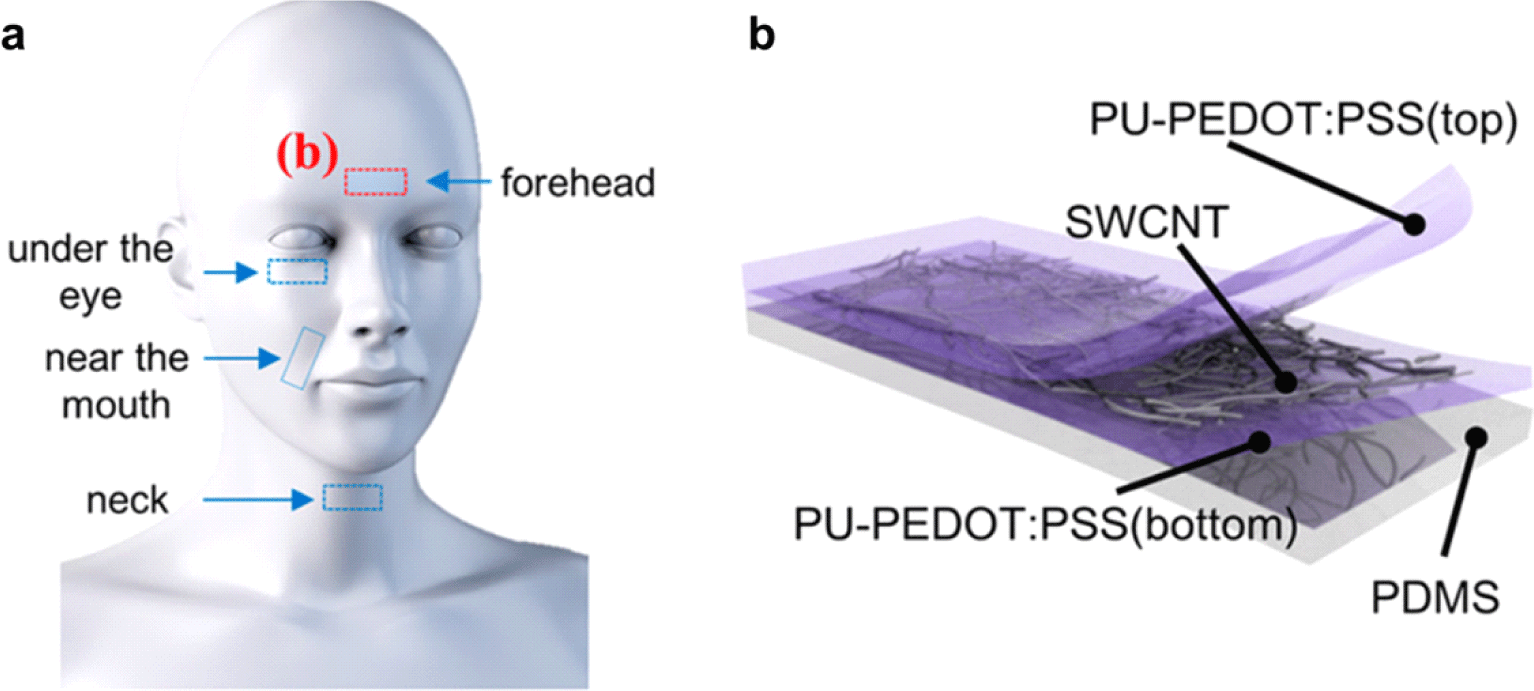
Jung et al. [34] developed a high-performance intrinsically soft conductor with exceptional conductivity, nanoscale thickness, and excellent stretchability—features that were previously unattainable with conventional methods—by pioneering the float assembly method, the first of its kind globally. The float assembly method involves three steps: (1) dropping a mixture of silver nanowires, an elastomer, and ethanol into a water-filled container, (2) adding a surfactant, and (3) drying the solvent (Fig. 9). The resulting material demonstrated an electrical conductivity of 100,000 S/cm, comparable to metals. It also retained its electrical properties without mechanical failure even when stretched to 10 times its original length. Additionally, with a thickness of only 250 nm—about 1/300th the thickness of a human hair—it could form complete contact with curved surfaces, such as skin. The research team used this flexible conductor to create a skin-attachable multifunctional layered device, capable of simultaneously monitoring various biometric signals, including electromyography, humidity, temperature, and tensile force, directly from the skin.
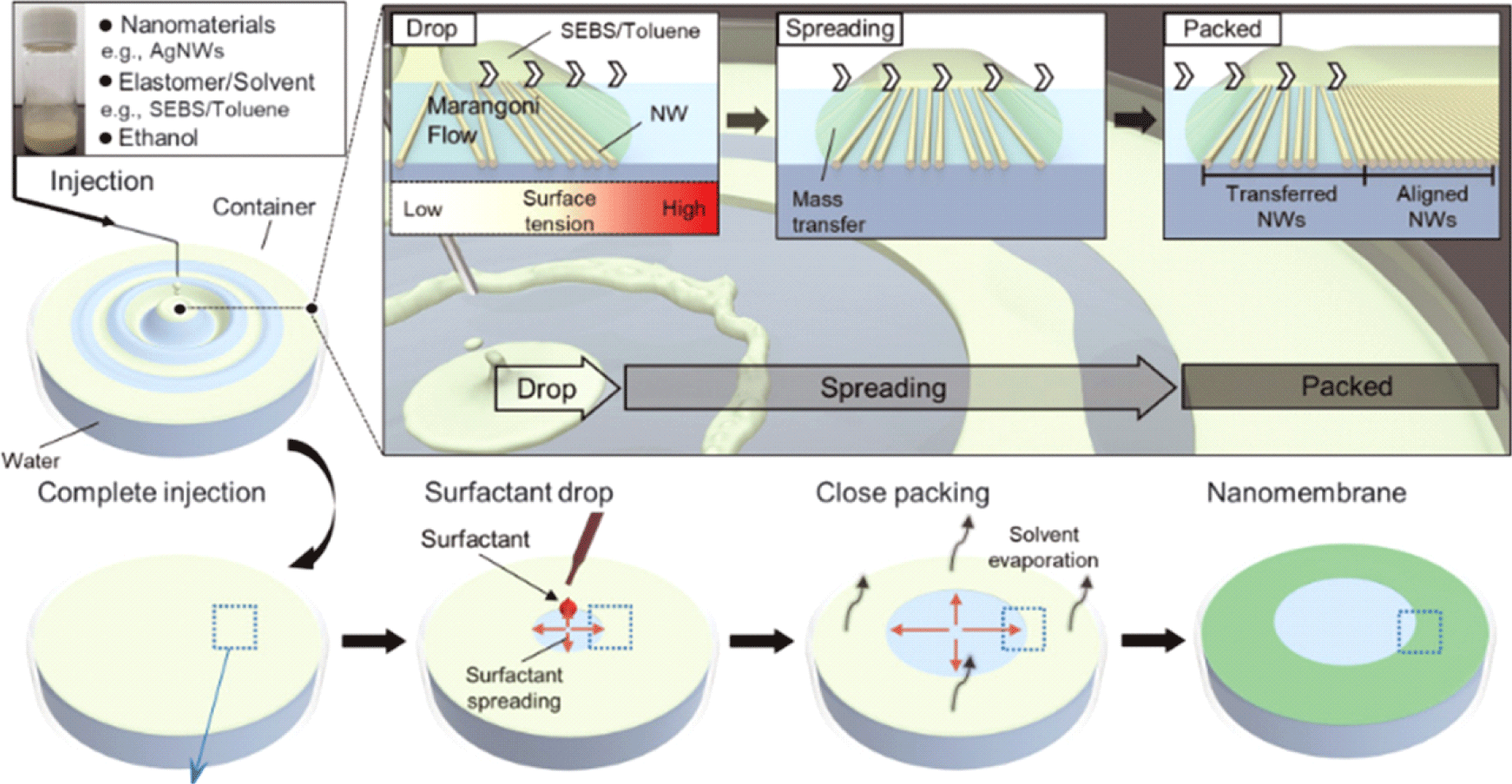
Research on intrinsically soft semiconductors is similarly focused on improving the electrical performance of the materials while maintaining their stretchability. However, unlike conventional inorganic materials with crystalline structures, polymers and semiconducting nanomaterials face inherent limitations in their electrical performance, making further enhancements challenging. Additionally, performance degradation caused by material fatigue under repeated mechanical deformation poses a critical issue. This has led to extensive research efforts aimed at overcoming these challenges and improving the reliability and durability of these materials.
Xu et al. [23] from Stanford University, led by Professor Zhenan Bao of the Chemical Engineering Department, developed an intrinsically soft semiconductor material with exceptional mechanical properties by leveraging the nanoconfinement effect observed when mixing the organic semiconducting polymer poly(2,5-bis(2-octyldodecyl)-3,6-di(thiophen-2-yl)diket-opyrrolo[3,4-c]pyrrole-1,4-dione-alt-thieno[3,2-b]thiophen) (DPPT-TT) with the elastomer polystyrene-b-lock-poly(ethylene-ran-butylene)-block-polystyrene (SEBS) (Fig. 10). The research team discovered that the nanoconfinement effect causes polymer aggregation, which increases the elastic modulus and delays the formation of cracks under strain. As a result, they reported that the semiconductor material's carrier mobility remained largely unaffected even when the material was stretched by approximately 100%. Using this material, the team fabricated intrinsically soft transistor devices and demonstrated that the carrier mobility of these transistors was comparable to that of amorphous silicon transistors. Furthermore, they confirmed that the transistors maintained their performance even when stretched in all directions. To showcase the material's capabilities, they conducted a demonstration where the transistor was used on skin to power a light-emitting device.
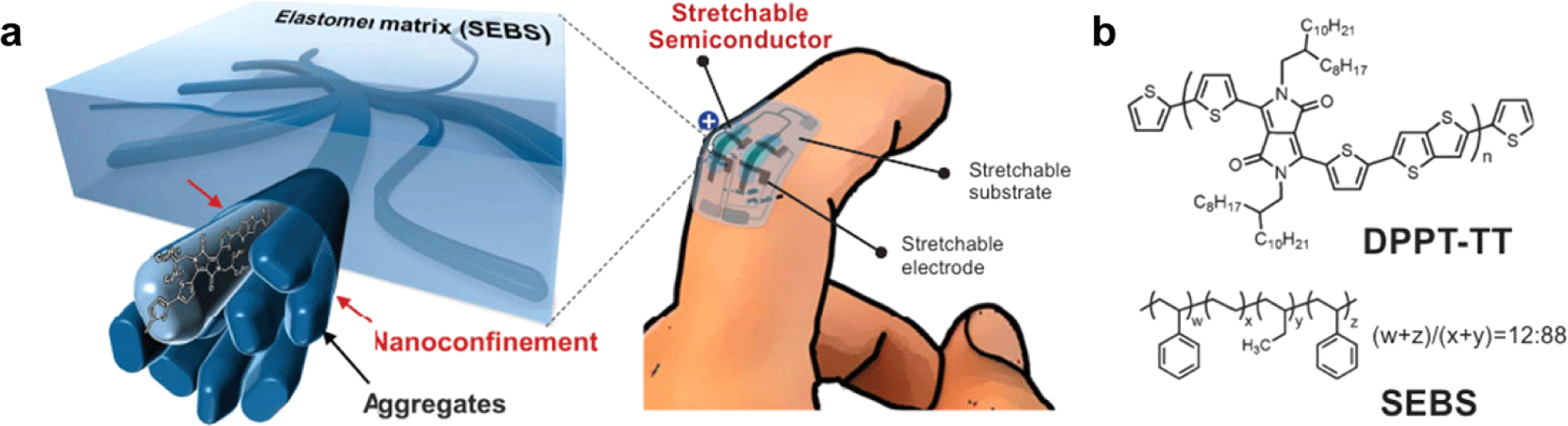
The following year, Zheng et al. [35], from the same research team at Stanford University, developed an intrinsically soft semiconductor material based on the organic semiconducting polymer indacenodithiophene-co-benzothiadiazole (IDTBT) and applied it to intrinsically soft transistor devices using a similar approach. The key difference compared to DPPT-TT-based intrinsically soft semiconductors lies in their structural properties. While DPPT-TT exhibits a semi-crystalline nature, which offers excellent electrical performance, it suffers from significant mechanical degradation when stretched beyond 100%, with cracks forming that cannot be reversed. In contrast, IDTBT, with its amorphous structure, is structurally more stable and does not experience performance degradation even when stretched beyond 100% (Fig. 11). Furthermore, the carrier mobility of IDTBT is comparable to that of DPPT-TT, maintaining a high performance of 0.6 cm2V−1s−1 even when stretched over 100%. The research team emphasized the use of durable organic semiconducting polymers to slow the accumulation of material fatigue, thereby addressing the limitations of intrinsically soft electronic devices.
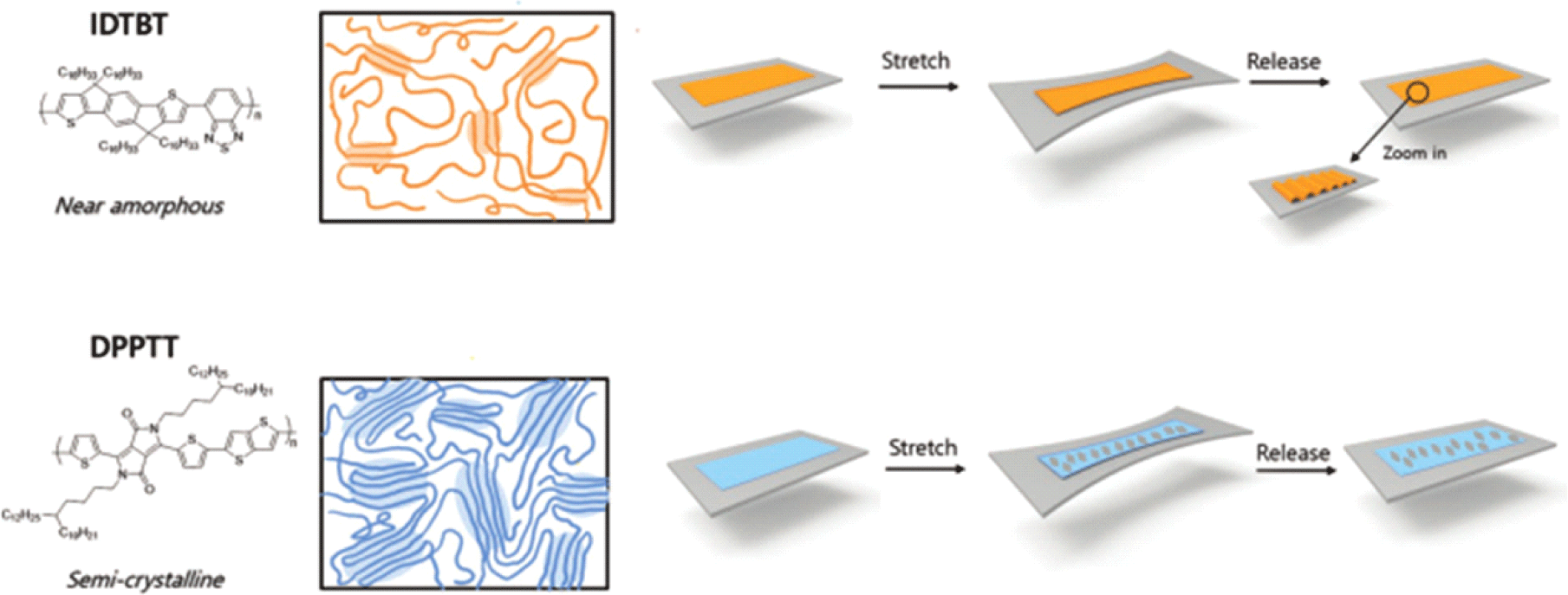
Song et al. [36] developed an intrinsically soft semiconductor material by optimally blending quantum dots, organic semiconducting polymers, and elastomers. This material effectively absorbs light across various wavelengths and maintains its performance even under physical deformation. The research team focused on the phase separation phenomenon occurring within the elastomer between the quantum dots and the organic semiconducting material when the three components were mixed in optimal ratios (Fig. 12). They discovered that as the material stretches, the spacing between the quantum dots increases, leading to a decline in electrical performance. However, they resolved this issue by using the organic semiconducting material to fill these gaps, enabling the material to convert light into electricity stably even under strain. Additionally, the team applied artificial intelligence (AI) and machine learning techniques to overcome the physical limitations of intrinsically soft semiconductor materials and enhance their photoelectric performance stability. By compensating for performance degradation caused by material fatigue and optical performance loss due to physical deformation, they improved the accuracy of a photodetector device fabricated using this material. This study is the first to introduce AI-based methods to address the limitations of intrinsically soft materials and electronic devices. The team expected this technology to be widely applied in the future for applications such as artificial retinas and wide-angle cameras.
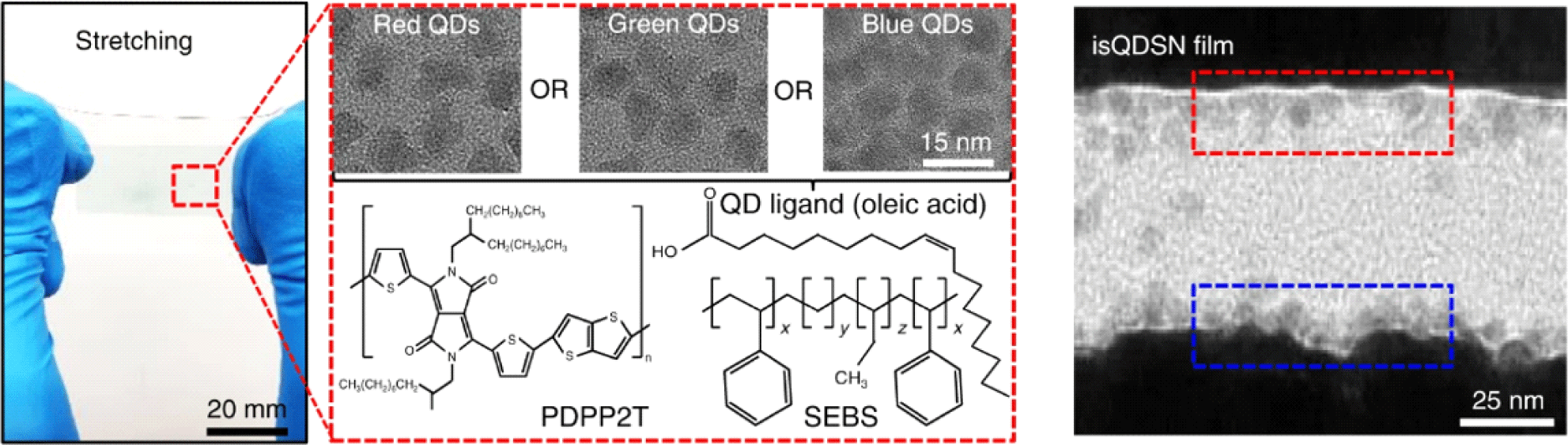
Despite advancements in conductor and semiconductor materials for intrinsically soft electronic devices, the performance of such devices reported to date remains significantly inferior to that of conventional electronic devices. Much of the research in this area has been at the proof-of-concept stage. The primary reason is the difficulty in improving the mechanical and physical properties of dielectrics while maintaining their insulating performance. Unlike the other two material types, dielectrics have seen less research activity and are mostly based on polymer materials that can only be deposited in liquid form. These materials typically have low dielectric constants, are challenging to control in terms of thickness, and are incompatible with conventional semiconductor fabrication processes, limiting the performance improvements of intrinsically soft electronic devices. One critical issue is that the operating voltage of most reported intrinsically soft transistor devices is extremely high, often ranging from tens to nearly a hundred volts. This makes them highly unsuitable for applications that require attachment to or insertion into biological tissues for purposes such as biometric signal collection, diagnosis, or therapy.
Koo et al. [37] developed the world's first nanoscale-thin and chemically/thermally stable ultrathin intrinsically soft insulating material that can be fabricated using vacuum deposition. Based on this, they created wafer-scale, large-area intrinsically soft electronic devices compatible with photolithography processes used in conventional semiconductor manufacturing. The research team utilized initiated chemical vapor deposition (iCVD) to develop the intrinsically soft insulating material. By optimizing the deposition process with an appropriate mixture of monomers and crosslinkers, which contribute to the material's softness and deformation properties, they fabricated a polymer ultrathin film with excellent stretchability and insulation properties. This film maintained a uniform composition even at a thickness of 100 nanometers (Fig. 13). The developed flexible insulating film exhibited a high breakdown voltage of 2.3 MV/cm and retained up to 40% tensile deformation capacity when repeated, even at a thickness of approximately 160 nm. It also demonstrated durability in thermal processes at around 300?, ensuring compatibility with traditional semiconductor device fabrication protocols. With these outstanding properties, the research team overcame previous technical barriers that had prevented stable insulation performance and deformation characteristics in films thinner than the micron scale. They successfully implemented intrinsically soft electronic devices that operate reliably at thicknesses below 200 nm. These devices achieved high capacitance and low-voltage operation due to the ultrathin design, resulting in the highest output current for the same channel area. This breakthrough in technology is expected to serve as a foundational platform for the large-scale integration of low-power intrinsically soft electronic systems. It has the potential to advance next-generation applications, including highly sophisticated artificial electronic skin and portable digital healthcare devices, making it a key enabling technology for future innovations.
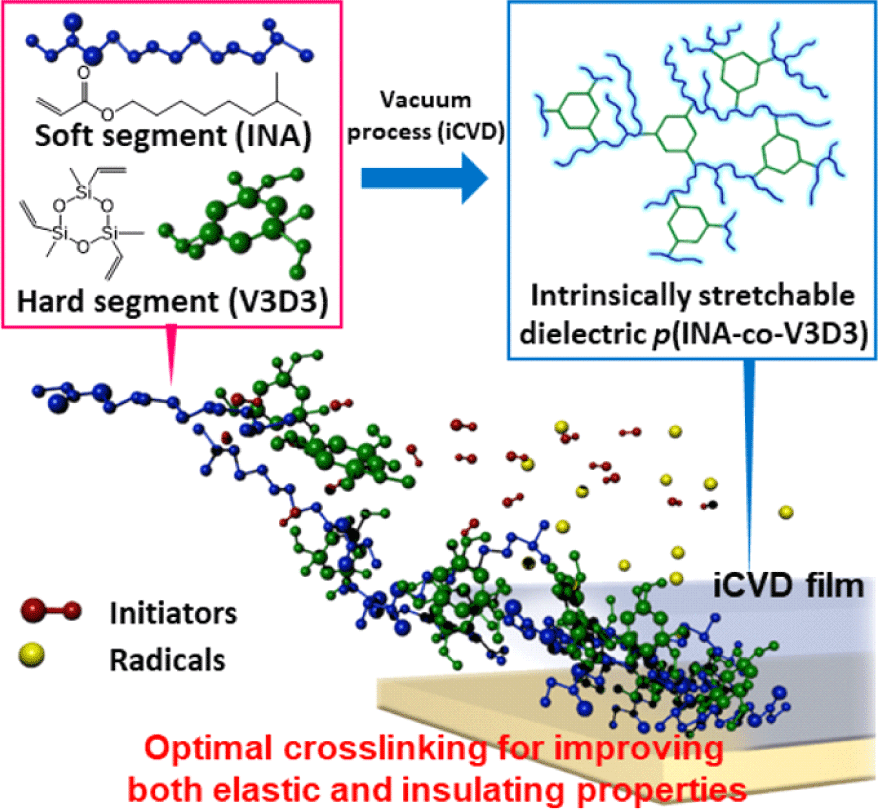
3. PERSPECTIVE AND FUTURE PROSPECTS
Soft electronic device technologies for digital healthcare have garnered significant attention over the past decade, with extensive research being conducted from various perspectives. Notably, if electronic devices are not soft, they are unlikely to function properly when attached to or implanted in body organs or tissues due to the mechanical mismatch between the device and the biological tissues. This mismatch can not only impair the performance of the device but also cause harmful effects on the body, making it difficult to achieve the intended purpose. Therefore, it is crucial to make electronic devices soft so that their physical and mechanical properties align with those of the organs or tissues they interact with. To achieve this, advanced soft electronic materials and stretchable device technologies must be developed. Intrinsically soft electronic devices, in particular, are gaining attention as key components for building next-generation digital healthcare systems. By using soft materials for all components of the device, these devices facilitate easier physical property adjustments and durability improvements.
This review article has introduced the latest developments in conductors, semiconductors, and insulators used in the fabrication of intrinsically soft electronic devices. However, the technology for fabricating intrinsically soft electronic devices remains in its early stages and is not yet fully mature. From commercialization point of view, the processing of soft materials mostly relies on solution-based processes, which are not compatible with the current vacuum-based microfabrication techniques. Development of soft conductors, semiconductors, and insulators which exhibit electrical performances comparable to those of the rigid materials still remains unaccomplished. Even if the materials are developed, their device integration strategies need to be investigated, along with their bio-integration strategies. Lastly, significant trial and error and close collaboration with researchers in the medical field are required before these technologies can be applied to digital healthcare applications. Nevertheless, meaningful progress is accelerating, and there is hope that digital healthcare prototypes based on intrinsically soft electronic device technologies will be launched in the market in the near future.